Semiconducting Metal Chalcogenide Aerogels
Semiconducting metal chalcogenide (say “kal-kah-jih-nide”) aerogels are a new, exciting class of aerogels materials. First prepared in 2002, these aerogels possess a unique combination of porosity, optical translucency, and photoluminescence, and show great promise for use as chemical sensors (artificial nose?) and energy applications such as photovoltaics (solar cells) and extraction of hydrogen from water using sunlight as the energy source.
Like other aerogels, metal chalcogenide aerogels consist of a network of interconnected nanoparticles which form a sponge-like, open-celled, nanoporous framework. But it is the carefully-engineered nanoparticles used to make these aerogels that makes them so special.
Before we dive into metal chalcogenide aerogels, below is a little bit of background on metal chalcogenides, semiconductors, and quantum dots. If you’re comfortable with the stuff already, skip down to the Metal Chalcogenide Aerogels section below.
Background on Metal Chalcogenides, Semiconductors, and Quantum Dots
What the Heck is a Metal Chalcogenide?
A metal chalcogenide is a compound made from a metallic element and a member of the chalcogenide family, namely the elements under oxygen–sulfur, selenium, and tellurium. Generally speaking the term metal chalcogenide isn’t really used to refer to metal oxides, however, although technically speaking metal oxides are metal chalcogenides. Metal chalcogenides of interest include cadmium sulfide (CdS), cadmium selenide (CdSe), cadmium telluride (CdTe), zinc sulfide (ZnS), and lead telluride (PbTe).
An example of a common application of a metal chalcogenide is cadmium sulfide (CdS) photoresistors, little devices which change their resistance based on the amount of light they are exposed to. Such cells are used for automatically turning lights on and off at night and are even available at Radio Shack.
Why Are Metal Chalcogenides Cool?
Metal chalcogenides are becoming increasingly important as semiconductors because they can be turned into quantum dots easily (see below) and because they can be made cheaply at ambient or close to ambient conditions.
One application is the production of advanced, narrow-wavelength (pure-color) LEDs for flat-panel displays. Quantum dots made from metal chalcogenides have extremely narrow emission spectra.
The biggest impact metal chalcogenides may have, however, is in solar power. Cheap, flexible solar panels produced in continuous rolls (as opposed to one batch of wafers at time) are just now (2008) becoming commercially available, and many of these technologies rely on metal chalcogenides as the photovoltaic material (the part that converts sunlight into electricity) because of their optimal combination of a decent conversion efficiency with the ability to be deposited at ambient conditions. This makes cheap, roll-to-roll manufacture possible.
Semiconductors for LEDs and Solar Cells: Understanding the Bandgap
Semiconductors have a property called bandgap (or energy gap). A semiconductor’s bandgap corresponds to the minimum voltage you have to apply to the semiconductor crystal before it will conduct electricity. From a hand-wavey-physics perspective, it refers to the gap between the lower energy states electrons are allowed to hang out in normally without thermal or electrical excitation (the valence band) and the higher energy states where electrons can move around and conduct charge through the semiconductor (the conduction band). This is different from metals and other conductors which will conduct electricity regardless of how small the voltage applied to it is.
If a semiconductor is used to make a photovoltaic device (like a solar cell), the bandgap corresponds to wavelength of light required to get the solar cell to generate electricity. This is because only photons of light with specific energies (colors) that happen to match the energy of the bandgap can excite electrons from their lower energy “hang-out” states (valence band) to their higher energy “let’s get up and move around” states (conduction band). In fact if you put a typical silicon solar cell like the kind you can buy at Radio Shack under a fluorescent light, it will produce virtually no current, whereas if you put it under bright incandescent bulb or in the sun it will. Simply put, the fluorescent light doesn’t output enough light with the right wavelengths to excite the bandgap of the semiconducting silicon.
If a semiconductor is used to make an LED (which you can also buy at Radio Shack), the wavelength (color) of light the LED will be emitted when a voltage is applied to it corresponds to the energy of the semiconductor’s bandgap. In the case of an LED, electric potential (voltage) is used to kick electrons up to their conduction band, and later when the electrons spontaneously jump back down to the valence band they release their extra energy in the form of photons matching the energy of the bandgap they are jumping over, and photons with a specific color are emitted.
Quantum Dots
Quantum dots are nanoparticles made of semiconductors which exhibit several amazing properties.
When a semiconductor crystal, made of silicon or a metal chalcogenide for example, is so small it is only a few nanometers in diameter, an effect called “quantum confinement” occurs. This effect arises from the fact that a nanoparticle contains only hundreds to thousands of atoms, as opposed to the billions upon billions of atoms of even the tiniest macroscopic crystal. Basically, the result of this effect is that the bandgap of the semiconductor widens as the nanoparticle shrinks.
A Little Bit of History on the Colors of Blinking Lights and Bandgap Engineering
The ability to change the bandgap of a substance simply by changing particle size is an amazing property! A little bit of history will help you to understand. For a long time, the only colors of LEDs available were a few specific flavors of red, yellow, and green. Like, for about two decades. That’s why all electronics between 1980 and 2000 have either red or green power lights on them. Even though blue LEDs had been made as early as 1971, the necessary semiconductor, gallium nitride (GaN), just didn’t give out enough light to make a practical LED. In the late 1980’s and early 1990’s, however, a breakthrough in the ability to make GaN and to modify GaN with dopants enabled high-brightness blue LEDs and, by the late 1990’s, blue LEDs started appearing in consumer electronics. Soon after, it was found that by using GaN and sandwiching/combining it with a few other semiconductors such as indium nitride (InN) and aluminum gallium nitride (AlGaN), production of LEDs with a wide spectrum of colors ranging from amber to ultraviolet become possible! And by combining blue LEDs with an embedded yellow phosphor, white LEDs then became possible!
But it took breakthroughs in semiconductor manufacturing and a little bit of re-measuring the bandgap of some exotic semicondutors (namely InN) before it became possible to make all of these colors.
Quantum Dots to the Rescue!
With quantum dots, we don’t need to rely on happening to find the right exotic substance with the right bandgap and figuring out how to manufacture that substance efficiently. Using just one semiconductor, we can tune that semiconductor’s bandgap just by making particles of the right size! For example, 2 nm CdS will emit blue light, 6 nm CdS will emit red light, and sizes in between will emit colors in between!
Furthermore, the colors quantum dots can produce are much purer than the colors produced by bulk semiconductors since there are many fewer allowed states and thus fewer possible emissions because of quantum confinement.
Metal Chalcogenide Aerogels
Newly appointed Professor Stephanie Brock at Wayne State University in Detroit, MI had the aerogel bug. She had been inspired by aerogels after hearing a talk by Dr. Debra Rolison from the Naval Research Laboratory who presented aerogels as an architecture for making materials such as batteries and chemical sensors and decided that when she had her own research group, aerogels were something she wanted to pursue, although initially she wasn’t quite sure what direction she wanted to go in.
Then one day, in thinking about the range of compositions of aerogels people had already made, she noticed that pretty much every type of aerogel, with the exception of organic and carbon aerogels, was some sort of metal oxide.
Where most people had been interested in switching the metal in these aerogels, Prof. Brock was interested in switching the oxygen.
“If you can make aerogels out of metal oxides, why not metal chalcogenides?” she wondered.
To answer this question, she then found herself upon the first question one asks when wondering if you can make an aerogel out of something. Can you first make suitable gels to start from?
To the literature Prof. Brock went and found that in fact recently metal chalcogenide gels had been made (T. Gacoin, K. Lahlil, P. Larregaray, and J-P. Boilot, Journal of Physical Chemistry B, 2001, 105, 10228-10235). So then she asked next the next logical question-can you supercritically dry such gels to make aerogels?
And so she did, and in the process, discovered an entire new class of aerogel materials.
In her paper to Science, Prof. Brock describes the work in her own words:
Mesoporous (pore size 2-50 nm) metal chalcogenides have been long sought because they blend desirable optical and electronic properties with a pore size that is optimal for rapid transport of gas-phase species. However, strategies commonly employed for oxides, such as surfactant templating for generation of ordered M41S-type oxides, result in structure collapse when the template is removed from mesostructured metal chalcogenides. Here we demonstrate that it is possible to create a series of stable mesoporous metal chalcogenides by oxidative cross-linking of nanoparticles to form a gel-body. Supercritical drying with CO2 permits access to the pores, creating a new class of aerogels based entirely on metal chalcogenide building blocks.
Aerogel formation has been demonstrated for diverse systems: CdS, CdSe, ZnS, and PbS, producing materials with surface areas as high as 250 m2/g (compared to a benchtop dried sample with a surface area of 55m2/g) with average pore sizes ranging from 15-45 nm. Unlike conventional silica aerogels and M41S-type materials, the framework is crystalline, consisting of quantum dots assembled into a pearl necklace-type morphology, and bulk monoliths exhibit the characteristic optical properties of these nanoscale building blocks — remaining quantum confined. Hence, the materials display sharp, band-edge photoluminescence at energies significantly blue shifted from single crystals. Post-annealing permits the absorption energy of the material to be tuned to the red as the framework coarsens and the average crystallite size grows.
The sol-gel methodology represents a powerful yet facile strategy for assembling metal chalcogenide nanoparticles into mesoporous functional architectures without compromising their unique size-dependent properties. The combination of high surface area, quantum confinement effects, and photoluminescence make metal chalcogenide aerogels potential candidates for photocatalytic, photovoltaic, and sensing applications. Current efforts are focused on tailoring (chemically or physically) these chalcogenide aerogels for desired applications.
Production of Metal Chalcogenide Aerogels
Just like the production of silica and metal oxide aerogels, the first step to making metal chalcogenide aerogels is doing some wet chemistry to make a gel, which means first making a solution of nanoparticles (a sol).
But unlike silica and metal oxide aerogels, there is no “mix-and-gel” chemistry where nanoparticles will both form and self-assemble nicely into a gel matrix for metal chalcogenides. Instead the process goes like this:
- Mix a bunch of chemicals together to start growing metal chalcogenide nanoparticles.
- Arrest nanoparticle growth by injecting a thiolate capping agent when they reach the desired size (if you don’t they will keep growing and agglomerating and eventually precipitate).
- Partially remove the capping agent by gently oxidizing it away with hydrogen peroxide.
- Allow the partially-decapped nanoparticles to assemble into a gel.
- Purify the gel by solvent exchange.
- Exchange gel into liquid CO2 and supercritically dry.
First Step: Make a Metal Chalcogenide Gel
Metal chalcogenide gels can be made through inverse micelle formation followed by arrested precipitation. In this technique, a water-based solution of metal salt, such as cadmium nitrate, is injected into a non-polar solvent, such as heptane, along with a surfactant. This forms little micro-pockets of water surrounded by surfactant molecules in the solution called inverse micelles, just like how soap solubilizes grease pockets in water. Next, a solution containing similar micro-pockets of chalcogenide-containing compound is injected. The two solutions of micro-pockets (called emulsions) mix, the micro-pockets break up and reform, and new micro-pockets containing mixtures of the metal salt and the chalcogenide compound form. Metal chalcogenide particles now form inside these micro-pockets. To stop their growth at the right size, a special capping agent is injected into the solution, which coats over the surface of the particles and prevents (arrests) them from growing any further.
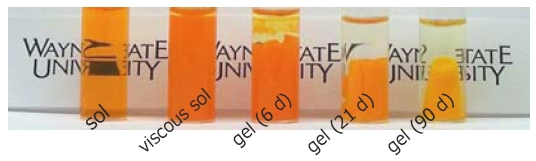
Assembly of a Gel from a Sol of Cadmium Sulfide Nanoparticles (image courtesy Prof. Stephanie Brock)
Below is an example of how CdS quantum dot gels can be made.
- Dissolve 111.14 g (0.25 mol) of di(2-ethylhexyl)sulfosuccinate sodium salt (a surfactant) in 500 mL of heptane (a non-polar liquid).
- A solution of aqueous cadmium nitrate, Cd(NO3)3, ranging from 0.01-0.2 M concentration, is added.
- The solution is stirred until clear. Surfactant-stabilized microsized pockets of the aqueous cadmium nitrate solution form. These pockets are called inverted micelles. The solution is called a microemulsion.
- A similar microemulsion of sulfide ions is prepared with sodium sulfide (Na2S). The two colloidal solutions are mixed and cadmium sulfide nanoparticles begin to form in the inverse micelles.
- Unused hydrogen sulfide is removed by bubbling nitrogen gas through the solution.
- A surface-capping agent, 4-fluorophenylthiol in triethylamine (a solvent), in the amount of five times the concentration of initial cadmium concentration, is added to the flask. This arrests the growth of the nanoparticles.
- The solution is centrifuged to precipitate out the nanoparticles.
- The nanoparticles are dispersed in acetone to a concentration of 0.05 to 0.5 M.
- Dispersed nanoparticles are gently oxidized by adding 0.1-0.2 mL of 3% hydrogen peroxide, H2O2.
- A gel forms. The gels are then purified and aged in pure solvent.
A similar procedure with the proper modifications can be used for ZnS, PbS, and CdSe. CdSe requires a different oxidizing agent, namely tetranitromethane.
References:
Jaya L. Mohanan, Indika U. Arachchige, Stephanie L. Brock*, “Porous Metal Chalcogenide Aerogels”, Science, Vol. 307, 2005.
T. Gacoin,† K. Lahlil,‡ P. Larregaray, and J-P. Boilot*, ‘Transformation of CdS Colloids: Sols, Gels, and Precipitates”, Journal of Physical Chemistry B, 2001, 105, 10228-10235.
Supercritical Drying
The metal chalcogenide gels are processed by typical Hunt process CO2 drying to produce metal chalcogenide aerogels.
Dots, Rods, and Tetrapods (Oh My!)
A huge amount of work has been put into the development of metal chalcogenide nanoparticles over the past decade, and because of this a wide variety of metal chalcogenide nanostructures are available. In fact, nanoparticles shaped like rods, barbells, and tetrapods, as opposed to spheres, can all be used to produce metal chalcogenide aerogels. Importantly, the morphology, surface area, and mechanical properties of aerogels produced with non-spherical particles is quite a bit different. Where spherical particles give a “string of pearls” morphology similar to silica and many metal oxide aerogels, other shapes give a fibrous morphology more characteristic of polymer aerogels. In the case of cadmium sulfide, the effect of the particle shape does this to surface area:
- Spheres (quantum dots), 110 m2 g-1
- Rods, 240 m2 g-1
- Branched Particles, 225 m2 g-1
- Tetrapods, 80 m2 g-1
Flavors of Metal Chalogenides Made So Far
The Brock group has produced aerogels of:
- Cadmium sulfide, CdS
- Cadmium selenide, CdSe
- Zinc sulfide, ZnS
- Cadmium sulfide/zinc sulfide composite, CdS/ZnS
- Lead sulfide, PbS
and using a different synthetic route,
- Germanium sulfide, GeSx
and many more!
Phosphides!
The Brock group has also extended the general technical for producing metal chalcogenide aerogels to phopshides, including:
- Nickel phosphide, Ni2P
- Manganese phosphide, MnP, and
- Indium phosphide, InP
These materials have totally different properties from the metal chalcogenides and represent an entirely different category of aerogels!
Properties of Metal Chalcogenide Aerogels
Metal chalcogenide aerogels exhibit a property called photoluminescence, that is, the glow when irradiated with a black light (ultraviolet lamp). In fact, metal chalcogenide aerogels have a bandgap, like other semiconductors, and that bandgap corresponds to the color of light they will emit under the black light. Bulk CdS has a bandgap around 2.05 eV (meaning the crystal will photoluminesce orange) but nanometer-sized CdS quantum dots can have a very different bandgap depending on their diameter. In general, aerogels made from quantum dots have about the same bandgap as the quantum dots their made out of, so CdS aerogels made from CdS quantum dots that have a bandgap of 2.73 eV (blue) will photoluminesce blue as well, although a bit greener of a blue (the bandgap of the aerogels is usually “red-shifted” compared to the quantum dots). Interestingly, if the aerogel is annealed at 300°C, the aerogel’s bandgap decreases to 2.22 eV (green). This means that the bandgap, and thus the photoluminescent color of metal chalcogenide aerogels can be tuned be heat treating!
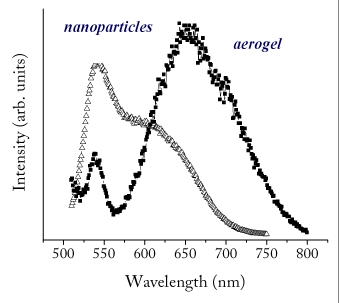
Photoluminescent Spectrum of Cadmium Selenide Aerogels vs. Unconnected Nanoparticles (figure courtesy Prof. Stephanie Brock)
Like other aerogels the density of metal chalcogenide aerogels is adjustable by adjusting the amount of solvent used to prepare the precursor gel, but typically metal chalcogenide aerogels have a density <0.1 g cm-3. This compares to a density of 1.7 g cm-3 for CdS xerogels (evaporated-dry gels). That means CdS aerogels are only 1.5% the density of bulk CdS! Interestingly, the bandgap and thus photoluminescent color of metal chalcogenide aerogels also changes with the density of the aerogel. The surface area of metal chalcogenide aerogels is somewhere around 100-250 m2 g-1, which may sound low compared to silica aerogels (~700 m2 g-1), but actually when you consider that the molecular weight of CdS is a lot heavier than silica, the surface area per mole is actually comparable to silica aerogels.
Although aerogels themselves are disordered materials, the particles in metal chalcogenide aerogels are crystalline in and of themselves. This gives rise to some interesting properties. The structure of metal chalcogenide aerogels also provides an interesting arena for understanding quantum confinement. Although the structure of the aerogel extends over three dimensions, it is actually made of fractal-like chains of particles, which is something like a one-and-a-half dimensional structure. Such a structure allows for scientists to think about the effects of quantum confinement in a different manner.
Applications of Metal Chalcogenide Aerogels
There are a number of exciting applications for metal chalcogenide aerogels. Most of these applications make use of the behavior of quantum dots in the aerogels while benefiting from the aerogels being macrosopic materials.
Photocatalysts
Using the right type of quantum dots, it may be possible to use metal chalcogenide aerogels to split water into hydrogen and oxygen using sunlight as the energy source. This is made possible through a phenomenon called multiple exciton generation, in which one photon causes multiple electron-hole pairs to form in the quantum dots (instead of one pair, which is what usually happens when a photon excites an electron across a bandgap). If a quantum dot aerogel can be tuned to generate four excitons per photon, hydrogen production from water and sunlight would become viable.
Photovoltaic Cells
Because the particles in metal chalcogenide aerogels are crystalline, they retain many of the semiconducting properties of bulk metal chalcogenides, including the ability to work as photovoltaic cells. Since metal chalcogenide aerogels can be made at ambient conditions and you can tune their bandgap by heat treating them, inexpensive solar cells based on metal chalcogenide aerogels could be optimally tuned to capture energy from multiple wavelengths from the sun.
Chemical and Biological Sensors
By attaching the right kind of chemical groups the surface of metal chalcogenide aerogels, you can potentially change their photoluminescent properties when exposed to certain chemicals or biomolecules, thus making a chemical or biological sensor. Since the photoluminscent properties and chemical compositions can both be tailored, these materials have a lot of possibilities for engineering sensors for specific applications.
Other Groups
Since Prof. Brock’s initial discovery, other groups have produced quantum dot metal chalcogenide aerogels including:
- Prof. Alexander Eychmueller’s group at the Dresden University of Technology (Technische Universität Dresden) in Dresden, Germany who demonstrated CdTe aerogels
- Prof. Mercouri Kanatzidis’s group at Northwestern University in Evanston, Illinois who demonstrated aerogels of germanium platinum sulfide (Ge4Pt2S9.6 and Ge4Pt2S8.7) and platinum tin selenide (Pt2.1Sn4Se9.7, Pt1.8Sn2Se5.7, Pt2SnSe4, and Pt1.4SnS4) through a totally different synthetic route where clusters of sulfide and selenide when bound to metal ions assemble into gels! These materials have high internal surface areas (up to 327 m2 g-1) and narrow bandgaps of 0.2-2.0 eV and may be useful for optoelectronics, photocatalysts, and removal of heavy metals from water.
Down the Road
Prof. Brock tells Aerogel.org that her group is currently exploring the applications of metal chalcogenide and phosphide aerogels for chemical sensors and energy applications. Of course who knows what other exciting aerogels will come out of the Brock group, as Prof. Brock is notorious for making stuff just because it seems cool.
For More Information
If you’re interested in learning more about metal chalcogenide aerogels, check out these articles.
On the Web
Scientific Papers
- J. L. Mohanan, S. L. Brock, “A New Addition to the Aerogel Community: Unsupported CdS Aerogels with Tunable Optical Properties,” Journal of Non-Crystalline Solids (special issue from the 7th International Symposium on Aerogels), 2004, 350, 1-8.
- J. L. Mohanan, I. U. Arachchige, S. L. Brock, “Porous Semiconductor Chalcogenide Aerogels,” Science, 2005, 307, 397-401.
- I. U. Arachchige, J. L. Mohanan, S. L. Brock, “Sol-gel Processing of Semiconducting Metal Chalcogenide Xerogels: Influence of Dimensionality on Quantum Confinement Effects in a Nanoparticle Network,” Chemistry of Materials, 2005, 17, 6644-6650.
- K. K. Kalebaila, D. G. Georgiev, S. L. Brock “Synthesis and Characterization of Germanium Sulfide Aerogels” Journal of Non-Crystalline Solids, 2006, 352, 232-240.
- J. L. Mohanan, S. L. Brock “CdS Aerogels: Effect of Concentration and Primary Particle Size on Surface Area and Opto-electronic Properties,” Journal of Sol-Gel Science and Technology, 2006, 40, 341-350.
- U. Arachchige, S. L. Brock “Sol-Gel Assembly of CdSe Nanoparticles to form Porous Aerogel Networks” Journal of the American Chemical Society, 2006, 128, 7964-7971.
- S. L. Brock, I. U. Arachchige, K. K. Kalebaila “Metal Chalcogenide Gels, Xerogels and Aerogels,” Comments on Inorganic Chemistry (invited contribution), 2006, 27, 103-126. Review
- I. U. Arachchige, S. L. Brock “Highly Luminescent Quantum Dot Monoliths” Journal of the American Chemical Society, 2007, 129, 1840-1841.
- I. U. Arachchige, S. L. Brock “Sol-Gel Methods for the Assembly of Metal-Chalcogenide Quantum Dots” Accounts of Chemical Research (invited contribution), 2007, 40, 801-809. Review
- Gaponik, Nikolai; Wolf, Andreas; Marx, Romy; Lesnyak, Vladimir; Schilling, Kristian; Eychmueller, Alexander “Three-Dimensional Self-Assembly of Thiol-Capped CdTe Nanocrystals: Gels and Aerogels as Building Blocks for Nanotechnology” Advanced Materials, 2008, 20, 22, 4257-4262.
- Bag, Santanu; Trikalitis, Pantelis N.; Chupas, Peter J.; Armatas, Gerasimos S.; Kanatzidis, Mercouri G. “Porous Semiconducting Gels and Aerogels from Chalcogenide Clusters” Science, 2007, 317, 5037, 490-493.